The Adaptive Continuum and How Species Succeed and Fail
Skip other details (including permanent urls, DOI, citation information)
SUBJECT TERMS
Article Type: Article
This work is licensed under a Creative Commons Attribution 4.0 International License. Please contact : [email protected] to use this work in a way not covered by the license.
For more information, read Michigan Publishing's access and usage policy.
Received 12 January 2018; Revised 30 September 2018; Accepted 19 October 2018
Abstract
Why do species fail to adapt? Implicit in this question is what happens to a single species that limits its adaptive potential and relegates it to a smaller niche than could otherwise be gained. One problem with this question is it views species or populations in isolation, as separate units, instead of as branches of a great tree of life. There are innumerable forms of life and speciation through natural selection is one important “origin of species.” Because selection is a strong driver of diversification, although just one of several drivers, speciation may often be adaptive. As species adapt and expand their niche they produce new branches, not unlike the life cycle of an organism. In this vein, the tree of life is partly an adaptive tree and has a niche formed from the accumulation of species. Adaptation is a niche expanding process, occurring at many evolutionary scales—an adaptive continuum—from genes to populations, species, and clades. Genetic variation is the fuel for adaptation, and at least three broad limits on genetic variation exist. First, time limits the building of genetic variation. Second, chance eliminates and constrains genetic variation through events such as catastrophes. Third, and underappreciated, speciation is both a limit and a solution to adaptation. Species lose genetic variation to the birth of new species, but the niche of the tree of life expands. Viewing species and all life in this way (an adaptive continuum) allows us to 1) reconcile the conundrum between the diverse tree of life and adaptive limits of individual species, 2) understand adaptation as a holistic process that includes processes below microevolution and above speciation and to frame research to understand the tipping point between adaptive micro- and macroevolution, and 3) develop approaches to biological conservation that look beyond single species and across the adaptive continuum.
Part of the special issue Species in the Age of Discordance, guest-edited by Matthew H. Haber and Daniel J. Molter.
1 Introduction
Why do species fail to adapt? This has been a long-standing question since Darwin posed it (1859), and is still often asked (e.g., Wiens 2004; Bridle and Vines 2007; Polechová and Barton 2015; Takahashi et al. 2016). How should we evaluate the adaptive success of an organism, and what is the relevant timescale to evaluate adaptation? Over a generation? Across the time span of a species? Here, I frame a perspective on the adaptive process and discuss how adaptation occurs and what factors affect adaptive potential. To provide a broad context for adaptation, I describe generalized species cycles that address how species originate, change over time, and give rise to new species, and I reason that there is an adaptive continuum with three main limits to adaptation: time, chance, and speciation. As a final goal, I identify a set of research problems that this view of adaptation brings to the fore in order to better understand adaptation and its limits.
What is adaptation? Adaptation can refer to modifications that benefit or advantage their owners, where “owners” refers to units under the influence of natural selection (Lloyd 2017). By this “engineering” definition (Lloyd 2017), adaptations provide better fit or performance in their environments and, importantly, can extend phenotypes beyond the prior range of variation. (When new phenotypes allow their owners to occupy new environments this is niche expansion—see section 3, Adaptive Continuum, below). Alternatively, adaptation may refer to modifications that are the result of a selection process; by this “selection-product” definition, adaptation reduces phenotypic range, and is thus not niche expanding and sometimes incompatible with the engineering definition (Lloyd 2017). In this article, I mainly use adaptation in the engineering sense.
What is an appropriate unit of selection, and at what biological level(s) should adaptation apply (Lloyd 2017)? It has long been argued that selection may act at many levels besides organisms within populations (Stegenga 2016). For example the principle of natural selection has been applied to species lineages (e.g., Hull 1980), to genes (e.g., Dawkins 2016), and even below genes to the molecular or atomic levels (Rosenberg 2006; Lloyd 2017). Hull (1980) distinguished between two kinds of units of selection: “replicators” and “interactors.” Replicators are entities whose structure is passed on directly through replication (Dawkins 1976), whereas interactors interact more directly with their environment (Lloyd 2017; Hull 1980; Gould and Lloyd 1999). The differential success of interactors determines the differential perpetuation of replicators housed within them or from where they originated. Interactors thus better constitute causal units of natural selection (i.e., Darwinian individuals). I take the viewpoint that units of selection can exist across multiple levels of organization (Sober and Wilson 1994), at sub- or supraorganismal levels (e.g., genes, demes, species, etc.)—i.e., a “hierarchical theory of selection” (Gould and Lloyd 1999). Such various units of selection can also be the units of adaptation. That is to say, if natural selection can happen at multiple levels, then so can adaptation, with caveats (see Gould and Lloyd 1999). While also addressing multiple scales of adaptation, I will focus my discussion primarily at the species level. I acknowledge that the term “species” may cause difficulty in light of discussions of their existence and definition (Ghiselin 1974; Mishler and Wilkins 2018) and discussions of phylogenetic discordance (Degnan and Rosenberg 2009). Nevertheless, species represent an important and distinctive biological scale or level for considering adaptation. Speciation, on many prominent views, is the process of evolving reproductive isolation (e.g., the biological species concept (BSC), Mayr 1942)—a viewpoint that I also espouse. For this reason, this project will focus on sexual reproducers. An adaptive continuum may certainly apply to asexual species, and to other species concepts, but these applications remain outside the current scope.
Life has been viewed as an ever-expanding tree going back to Darwin (1859), and while this continues to be a useful analogy, the history of life resembles a network more than a tree (Bapteste and Huneman 2018). If life adapts often at its branch tips, then the entire tree might be viewed as an adaptive tree (Doolittle 2017); that is to say, the same principles can apply to the tree as applies to the tips (species). Indeed, speciation, or lineage splitting is often thought of in adaptive light (Nosil 2012; Schluter 2000). This is not to say that all speciation or phenotypic variation is adaptive or shaped by natural selection (Gould and Lewontin 1979; Bapteste and Huneman 2018; Zhang 2018; Orzack and Forber 2017; Laland et al. 2015). Nevertheless, adaptation is common and perhaps inevitable in some manifestation if a species persists long enough to be influenced by selection (Darwin 1859; Hereford 2009; Sanford and Kelly 2011; Nosil 2012). Over billions of years, species of the tree of life have established an expansive reach across myriad ecological conditions (i.e., niche breadth). Viewed cumulatively, this expansive evolution of the niche breadth of life is in many ways an expression of adaptation.
To better understand how life adapts, and fails to adapt, requires a view of adaptation from a variety of biological scales at which genes, populations, species, and all of life operate. Viewing life and evolutionary or ecological processes at various scales along a continuum may be more profitable than across discrete levels of hierarchical organization (Potochnik and McGill 2012). Acknowledging this variety of scales invokes an adaptive continuum, described below, but first, I will discuss the sequence of development of a species (likening this process to the familiar notion of life cycles), which is important for understanding the context of adaptation.
2 Species Cycles
Once a species comes into being, and if successful, it will expand its range until it splits or produces new species or goes extinct, and this expansion and cladogenesis can be viewed as a cycle: the rise, reproduction, and decline of a species. Species cycles are analogous to an individual or organism life cycle (Hull 1976; de Queiroz 1999; Gould and Lloyd 1999), and there are long-standing traditions of viewing them in this manner (Rieppel 2011). Here I propose the stages birth, growth, and maturity (reproduction) (fig. 1). The life cycle analogy is convenient, but not strict, since species do not have a set ontogenesis. However, insights to viewing species in this manner are gained since both organisms and species cycle completely, from genesis to termination, and can transform in various ways (de Queiroz 1999). Just as life cycles differ greatly by type of organism, species cycles also differ. Species have a discrete birth or inception point when they come into being (Gould and Lloyd 1999). Applying the BSC, this birth point would occur when reproductive isolation has occurred sufficiently to separate or highly diminish gene flow from a progenitor (parent) species. In reality, the process of speciation is more of a continuum than a discrete process (Shaw and Mullen 2014). Although I adopt the BSC, I acknowledge arguments that reproductive isolation may not be necessary nor sufficient for speciation (de Queiroz 1999), and there are a variety of ways in which species may form. At this incipient stage of the species, low genetic variation within and among individuals may be present; there are of course exceptions as some species begin with large geographic ranges, having been separated from a progenitor species by a geographic barrier through allopatric speciation (Coyne and Orr 2004).
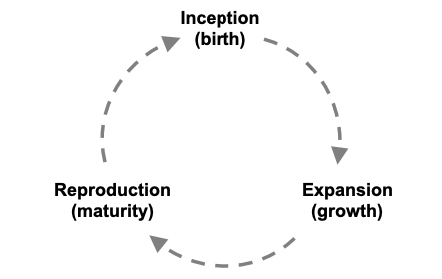
In the growth phase of a species, population expansion and correlated expansions of genetic variation through mutation and recombination occur. A range of possibilities exists for how much a species may expand and evolve or achieve “sufficient stability” (Gould and Lloyd 1999); some may grow very little or go extinct before expanding, whereas others may expand to cover continents. This increasing genetic variation leads to increasing adaptive differentiation and adaptive plasticity (Ghalambor et al. 2007), the products of drift and local adaptation through natural selection. During this phase, as populations expand and differentiate, the niche of the species—the set of all conditions in which a species can live and reproduce (Hutchinson 1957)—also expands. This niche expanding process is adaptive since changes in the species are conferring advantages that allow it to colonize and expand into new habitats and environmental conditions (e.g., Gomulkiewicz, Holt, and Barfield 1999). Adaptive expansions are referred to as secular migration (Lomolino, Riddle, and Brown 2005), implying gradual range expansions through iterations of dispersal and adaptation. Observing biological invasions, we witness how rapidly species distributions may expand in response to new ecological conditions given sufficient genetic variation (Lavergne and Molofsky 2007; e.g., Phillips et al. 2006). However, evolutionary novelties important in niche expansion and niche construction can also arise through environmentally induced variants (West-Eberhard 2005), and new research frameworks such as the “extended evolutionary synthesis” (Laland et al. 2015) are improving understanding of the importance of non-genetic inheritance in adaptation (Moczek et al. 2015). Species range limits arise when the necessary genotypes have not evolved to allow further expansion; if they do arise, the limits can expand. Furthermore, rare, advantageous alleles may promote expansion at the range boundary if they migrate there. As a species’ range expands, a genetic tension may arise from the challenge of occupying very different environments and maintaining gene flow. In this vein, prezygotic reproductive isolation can occur, creating population structure, and in some cases eventually resulting in speciation (e.g., peripatric speciation, Mayr 1982). For a progenitor species, isolated populations represent lost genetic variation and the potential birth of new species with future adaptive potential. Even if their origin is non-adaptive, new species are likely to adapt to future selection given sufficient genetic variation.
Reproduction in a species cycle occurs when species give rise to new species; i.e., cladogenesis (fig. 1). Although the topic of the geography of speciation has been rigorously debated (Coyne and Orr 2004), it is now generally appreciated that speciation can happen at an impressive variety of scales across a species’ geographic range, internally (sympatric) or externally (allopatric). Moreover, there are many modes and mechanisms of speciation, and it can occur at short time scales. When speciation results in phenotypes that can colonize new environments (i.e., niche expansion) the speciation process is arguably adaptive (e.g., as in ecological speciation). Taking the monkeyflowers as an example, the speciation process has been highly diverse (Wu et al. 2007), and has often resulted in niche evolution or expansion. Within one subclade of monkeyflowers alone, the yellow monkeyflowers (Section Simiolus), evidence for a great diversity of speciation and divergence modes and mechanisms has been observed (e.g., Cooley et al. 2011; Fishman et al. 2014; Garner et al. 2016; Martin and Willis 2007; Oneal, Willis, and Franks 2016). Case studies in the yellow monkeyflowers have included rapid, local adaptation and speciation on toxic soils (copper mine tailings) (Macnair 1989), allopatric and sympatric speciation through “budding” from small populations occupying different environments (Grossenbacher, Veloz, and Sexton 2014), and specialized, convergent evolution to unique habits (Ferris, Sexton, and Willis 2014). As with plants in general, speciation through auto and allo-polyploidy is common in this clade (Beardsley et al. 2004; Benedict et al. 2012; Sweigart, Martin, and Willis 2008). In the past few decades, rapid autopolyploidization in naturalized monkeyflower populations in the UK has been observed (Simón-Porcar et al. 2017) and even a new species has formed in the invaded range through hybridization and subsequent allopolyploidy (Vallejo-Marín et al. 2015). Monkeyflowers are not special in these regards—such contemporary observations of speciation through hybridization are becoming common in plants via human-caused biological invasions (Vellend et al. 2017). Rather, when a clade is examined closely we see that speciation occurs in many ways. The results of speciation are often adaptive; the clearest cases are “adaptive radiations” (e.g., Gillespie 2004; Jorgensen and Olesen 2001; McDonald and Kreitman 1991; Seehausen 2006). Thus, speciation can be considered a normal part of the adaptive process even in contemporary timescales as species respond to global change. Of course, how speciation and adaptation are related varies by lineage and its modes of generating genetic variation (e.g., gene transfer in bacteria, Thomas and Nielsen 2005). It is in this reproductive phase of a species cycle that a species may achieve its greatest adaptations, but perhaps by losing genetic variation to its offspring (discussed below).
Extinction represents an end point for a species and has naturally been viewed as a failure to adapt. Nevertheless, whether extinction represents a failure to adapt should be viewed in light of the species’ history and whether or not it has given rise to other species. When evaluating adaptive limits, we should first evaluate a species’ history and whether it may have generated offspring species. Did it arise as some type of specialist? Was it a vicariant offshoot from some other species, and how old is it? Perhaps it is expanding adaptively, but how would we know? Species are sometimes assumed to have a static niche when viewed across fossil records, but this may not be the case (DiMichele et al. 2004). When one considers all individuals and populations of a species, true niche stasis over long spans of time becomes doubtful. Anagenetic change may be adaptive (e.g., through stabilizing or directional selection), so even species maintaining a similar range or limit over long expanses of time may be considered adaptive. In this vein, we may generate alternative viewpoints on what constitutes an adaptive species and this can depend on scale. For example: a species that produces genetic variation contributing to adaptive differentiation (microevolution) or to the birth of new species (macroevolution).
Adaptive species expand the niche of the tree of life. Thus, adaptive species may give rise to new species, even if at the cost of their own extinction. Indeed, extinction rates appear to be positively correlated with speciation rates (Nixon and Wheeler 1992); lineages respond to rapid environmental change such as those seen during mass extinction events by splitting and diverging into new radiations. Janz and Nylin (2008) envisaged cyclic niche expansions and contractions (“oscillation hypothesis”) of phytophagous insects and their plant hosts, resulting in cladogenesis and radiations into new habitats. These are similar to species cycles. Organismal individuals transform during their life cycle (e.g., sexual transitions, life history changes), and species also transform such as in expansions, contractions, and subdivisions (de Queiroz 1999; Rieppel 2013). In considering species cycles, one is reminded of the fractal nature of the hierarchies of biological organization. Taxa at many levels (e.g., families, genera, species) are posited or interpreted as individuals when observed arriving and going from geological records, referred to as phylogenetic cycles. (Oskolski 2011). Similar to individuals and populations, entire clades may be under selection (Doolittle 2017), and if so, then it is logical that clades might also adapt. Theory on adaptation at large scales (e.g., clade selection) is controversial, as it is with smaller scales below populations (Lloyd 2017). Godfrey-Smith (2009, 6) offers one general solution: that adaptation through natural section should be applied to Darwinian populations, each defined as “a collection of particular things—that has the capacity to undergo evolution by natural selection.” How and whether to apply such definitions across all biological scales are open questions.
3 The Adaptive Continuum
Adaptation is a continuum (fig. 2). The process of improvement in the face of ecological challenges happens at many scales and stages. All life likely began as one population (Theobald 2010), perhaps one gene, and, viewing life as a single tree with many branches extending its ecological niche space, niche expansion seems to be mainly the result of myriad speciation events. Viewed broadly in this way, speciation may be the main adaptive process. Thus, when we consider limits to adaptation, we should mainly consider, what are the limits to speciation? That question addresses a large part of the adaptive capacity of life.
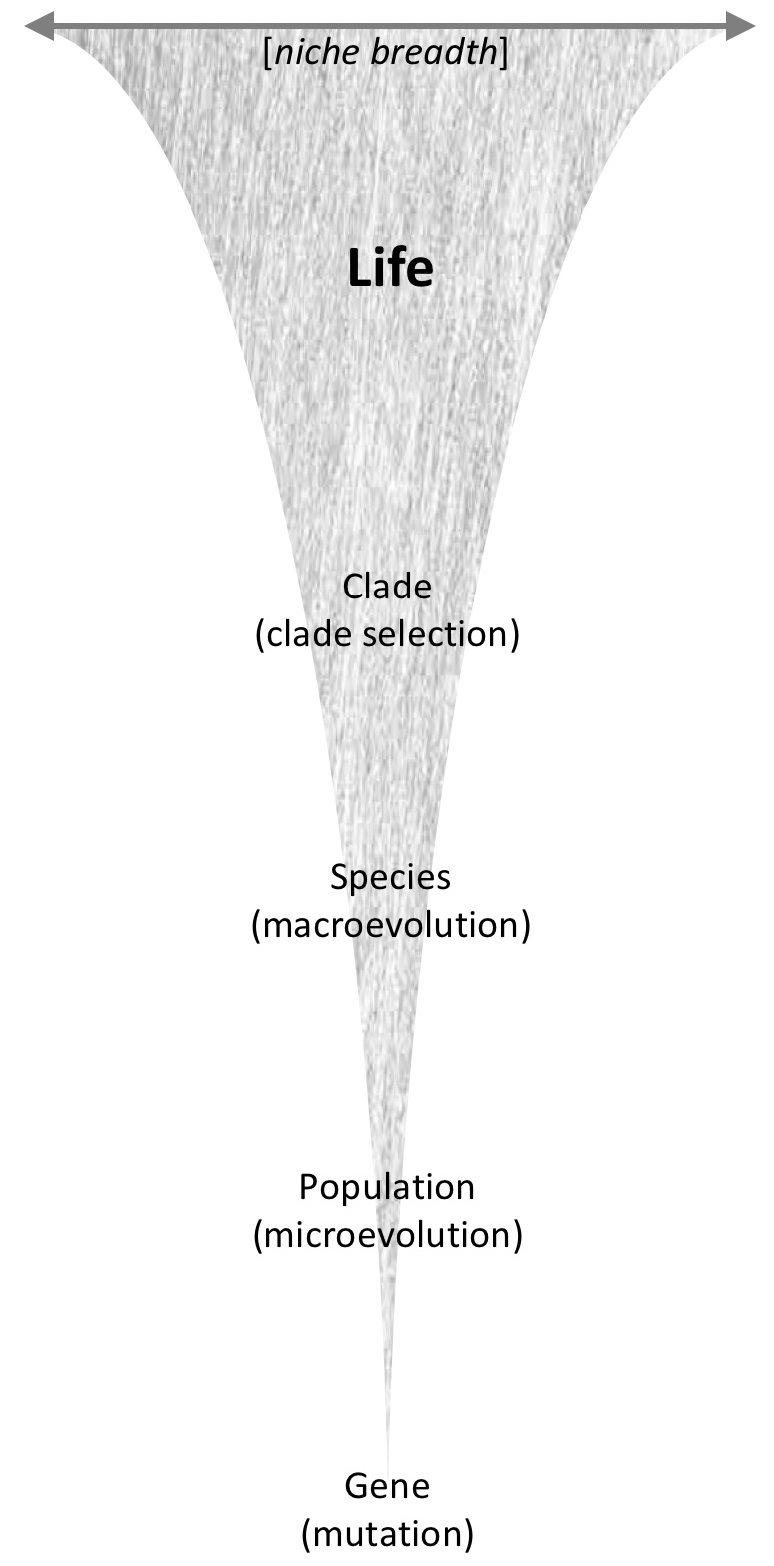
The adaptive continuum mirrors the process of differentiation and reproductive isolation among populations that leads to speciation, or equivalently the speciation continuum (Nosil 2012; Shaw and Mullen 2014). However, the adaptive continuum can include variation below populations and above species (fig. 2), and may include engineering and selection-product contexts described earlier (Lloyd 2017). Genes can mutate, altering their success. Whether clades are under the influence of natural selection is debatable (Doolittle 2017; Haber and Hamilton 2005; Hamilton and Haber 2006). Nevertheless, a clade’s attributes certainly determine its success (e.g., the great diversity of beetles compared to other insects; the extinction of non-bird dinosaurs at the end of the Cretaceous Period), and clades adapt in the sense that their attributes can change over time, which can make them better suited to their environment.
There are several considerations that should be made regarding the adaptive continuum and ecological niches. First, the adaptive continuum includes both microevolutionary and macroevolutionary adaptive processes. Microadaptation has traditionally been applied to adaptive changes among populations within a species (i.e., local adaptation), whereas macroadaptation refers to adaptive change associated with speciation. The former implies small changes among breeding individuals or populations, whereas the latter implies larger adaptive leaps. Both are cases of niche evolution. As niches evolve, so may niche breadth, the range of environmental conditions set by the niche, and which can be quantified at any phylogenetic scale in a biological hierarchy (Sexton et al. 2017). Second, niche evolution or pioneering may occur at practically any spatial or geographic scale depending on the organism. There are significant ecological gradients across small and large spatial scales and organisms may adapt to these gradients given sufficient genetic variation. Third, a population may be able to adapt, and to change phenotypically during the process, only so much before becoming reproductively isolated from other populations. This tension between niche evolution and reproductive isolation may be a major driver of ecological speciation (discussed in the next section).
4 Limits to Adaptation
Regarding adaptation as it applies to the expansion of ecological niche breadth, lack of genetic variation may be the ultimate limit to adaptation. This is clear for adaptive limits driven by a lack of heritable genetic variation within an individual’s genome from which natural selection can act (Conner and Hartl 2004). However, the genetic variation of interacting species is also important, and heritable in the case of endosymbionts (Laland et al. 2015). In considering adaptive biological interactions (e.g., symbiotic gut microbes, Bennett and Moran 2015), genetic variation in the form of genotype by genotype interactions is critical. Moreover, due to the fact that eukaryotes are in reality host to an extremely diverse microbiome, together making hologenomes, it has been argued that animals or plants should really be considered “biomolecular networks” (Bordenstein and Theis 2015). Even in cases of environmentally driven phenotypic plasticity, intra- or intergenic variation, the genetic machinery that alters phenotypes through gene expression, and the microbiome likely interact for phenotypes to respond adaptively to environmental cues. Thus, we should consider the limits to adaptation to really be limits on the generation and interaction of genetic variation within and among genomes. I propose three broad categories of adaptive limitation on genetic variation: time, chance, and speciation.
Regarding time as a limit, it takes time to build genetic variation, and time contributes to the buildup of genetic variation in many ways. It takes time to increase population sizes and for individuals to distribute or disperse to different environmental arenas of natural selection where adaptation occurs. Of course, it takes time for mutations to build and for recombination to produce new variants. Timescales of adaptation vary remarkably across different life histories, and species with short generation times are less limited by time. A familiar contemporary example is antibiotic resistance in microbes—something that can evolve in a matter of weeks (Palumbi 2001).
Second, adaptation can be limited by chance. Populations are often evolving; yet ecosystems are also often changing, driving adaptation and splitting and diverging populations. There are many ways in which chance can operate to limit or enhance adaptation, including catastrophes, environmental shifts, and species interactions. Returning to the prior example, the Cretaceous-Tertiary extinction ended the dinosaurs, excepting birds, but spurred the adaptive radiation of mammals (and birds). All mass extinctions have involved some form of environmental shift and differ in their pace of change. In this same vein, environmental shifts can be gradual, rapid, global, or regional, and the likelihood that these shifts will limit adaptation is relative to the available genetic variation and rate of environmental change. Similarly, biological interactions can be positive, negative, or neutral, but they certainly influence the adaptive trajectory of a species. Biological interactions are dynamic across ecological and evolutionary time scales. A predator, mutualist, or endosymbiont can appear, disappear, or reappear to interact with a given species and these shifting interactive dynamics may destroy genetic variation by increasing selection pressures, or stimulate new genetic variation by creating new adaptive opportunities (Laland et al. 2015). Thus, which species are encountered and what their net effect will be on adaptation is a matter of chance. Myriad species interactions among close and distant relatives influence the adaptive direction and expansion of lineages, ultimately shaping niche evolution and niche breadth across the tree of life.
Finally, speciation is both a limit (to ancestors or progenitors) and a major process of adaptation. As a limit to adaptation, speciation represents losses in genetic variation from a progenitor species to an offspring species. (I acknowledge that species often later form new adaptive combinations through hybridization—e.g., allopolyploid speciation in plants.) For example, consider a case of ecological speciation in which a new species has budded off—perhaps a new plant species pioneers a stressful soil type and establishes reproductive isolation. In the process of evolving adapted forms, new species bud off, removing that newly carved niche space, and the associated genetic variation required to be successful in it, from the parent species. Viewing the parent species only, this expansion into new environments (niche expansion) by cladogenesis appears as niche stasis in the fossil record. In understanding speciation as a limit, we must consider the relationship between niche evolution and reproductive isolation. To expand across very different environments often requires speciation. That is to say, niche divergence appears to be greater between species (or lineages) than within species (or lineages), which is the general principle of phylogenetic niche conservatism (Wiens and Graham 2005; Pyron et al. 2015). In this vein, a general positive correlation between niche divergence and reproductive isolation is likely to be a driver of adaptive biological diversification. Speciation is often viewed as a continuum (Nosil 2012; Shaw and Mullen 2014), and reproductive isolation correspondingly strengthens as populations diverge. It is apparent that through ecologically or geographically caused isolation and selection, populations will genetically adapt to varying conditions. Nevertheless, a species can only vary phenotypes so much and be able to maintain reproductive connectivity. Great leaps in niche evolution require changes in form, function, or reproductive timing, which give rise to reproductive isolation. At some point, gene flow between disparate ecologies or geographies will be too weak to maintain a biological species, although reproductive compatibility differs greatly between species at the outset of speciation (Gourbière and Mallet 2010). Thus, a species fails to adapt across all ecological gradients because its populations cannot evolve to occupy all environments and simultaneously maintain reproductive compatibility with all other populations of the species. Considering the adaptive continuum, it is not surprising that speciation happens before a taxon can adapt to all environments. There are too many potential reproductive barriers, prezygotic and postzygotic, that can accrue to see the evolution of a species that is the master of all environments. Nevertheless, although the loss of significant genetic variation from an original owner (progenitor species) to a descendant species may limit that progenitor, speciation events represent adaptive expansions for the tree of life.
5 Future Research Areas
Viewing adaptive limitations as constraints of time, chance, and speciation across an adaptive continuum raises several questions for future research:
- What determines the tipping point between adaptive micro- and macroevolution? That is to say, which conditions promote local adaptation (gradual niche expansion) versus ecological speciation (niche pioneering), both ecologically and genetically?
- Do the genomics of microevolution and macroevolution differ, and if so, how? What are the genomics of increasing adaptive plasticity? Understanding the genetics of how organisms expand their adaptive plasticity to increase niche breadth would shed light on the conditions that result in local adaptation within species or ecological speciation between species.
- Is there greater adaptive novelty (niche divergence) in areas where reproductive isolation and differences in natural selection are greatest (e.g., species range limits; islands)? That is to say, is there a geography of novelty or adaptive innovation (genetic variation) (e.g., Lesica and Allendorf 1995), or geographic zones of tension between local adaptation and speciation?
- Does climate change create more frontiers or opportunities for species to pioneer? That is to say, will speciation be required in many cases to adapt to contemporary climate change? If so, how should humans as ecosystem managers maximize this adaptive potential?
- Related to this last point, how much can a species’ niche expand or adapt to contemporary climate change? Gene flow or hybridization may expand a niche (i.e., transgressive segregation), but how much adaptive trait change is possible (see Rieseberg, Archer, and Wayne 1999)?
- Regarding the conservation of biodiversity and its adaptive capacity, how do we best conserve phylogenetic novelty and endemism (e.g., Thornhill et al. 2017)? In order to conserve diversity, we must conserve evolutionary capacity (including speciation), and understanding the tension between adaptation and speciation is important. For instance, although assisted migration or prescriptive gene flow may be an option to increase adaptive capacity (Smith et al. 2014), it should not swamp out populations that may be undergoing speciation.
6 Conclusion
“Why do species fail to adapt?” is an important question—and it should be framed within shallow and deep evolutionary time. Species have often failed and often not failed to adapt. Sometimes the successes have extended ecotypes of a species across continents. Sometimes the successes have resulted in adaptive speciation, expanding the clade, or broadly speaking, the tree of life. How far can populations expand their niches before they split into new populations, or, when does adaptation lead to divergence? Such questions or research programs should consider an adaptive continuum, from genes to species, and above. Viewing life as an adaptive continuum incorporates the tree of life into our thinking on adaptation and may offer several benefits: 1) It reconciles the conundrum of single species limits within a vast tree of life. That is to say, it addresses the problem of why populations and species appear to be so limited by genetic variation, yet there are millions of diverse genetic forms (species). 2) It offers a view of adaptation as a holistic process, from genes to lineages, including what we think of as micro- and macro-evolution. 3) It focuses investigations to understand the relationship between niche evolution and reproductive isolation during speciation. And 4) It may improve conservation and restoration of biodiversity and ecosystems by including holistic thinking below and above the species level. With the sixth mass extinction, humans are altering the niche of the tree of life; it is important that we consider the entire adaptive continuum in our goals to promote resilience and reduce vulnerability of Earth’s ecosystems.
Literature cited
- Bapteste, Eric, and Philippe Huneman. 2018. “Towards a Dynamic Interaction Network of Life to Unify and Expand the Evolutionary Theory.” BMC Biology 16: 56. doi:10.1186/s12915-018-0531-6.
- Beardsley, Paul M., Steve E. Schoenig, Justen B. Whittall, and Richard G. Olmstead. 2004. “Patterns of Evolution in Western North American Mimulus (Phrymaceae).” Am. J. Bot. 91 (3): 474–89. 10.3732/ajb.91.3.474.
- Benedict, Beverly G., Jennifer L. Modliszewski, Andrea L. Sweigart, Noland H. Martin, Fred R. Ganders, and John H. Willis. 2012. “Mimulus sookensis (Phrymaceae), a New Allotetraploid Species Derived from Mimulus guttatus and Mimulus nasutus.” Madroño 59 (1): 29–43. doi:10.3120/0024-9637-59.1.29.
- Bennett, Gordon M., and Nancy A. Moran. 2015. “Heritable Symbiosis: The Advantages and Perils of an Evolutionary Rabbit Hole.” Proceedings of the National Academy of Sciences 112 (33): 10169–76. doi:10.1073/pnas.1421388112.
- Bordenstein, Seth R., and Kevin R. Theis. 2015. “Host Biology in Light of the Microbiome: Ten Principles of Holobionts and Hologenomes.” PLOS Biology 13 (8): e1002226. doi:10.1371/journal.pbio.1002226.
- Bridle, Jon R., and Timothy H. Vines. 2007. “Limits to Evolution at Range Margins: When and Why Does Adaptation Fail?” Trends in Ecology & Evolution 22 (3): 140–47. 10.1016/j.tree.2006.11.002.
- Conner, Jeffrey K., and Daniel L. Hartl. 2004. A Primer of Ecological Genetics. Sunderland, Massachusetts: Sinauer Associates.
- Cooley, Arielle M., Jennifer L. Modliszewski, Megan L. Rommel, and John H. Willis. 2011. “Gene Duplication in Mimulus Underlies Parallel Floral Evolution via Independent Trans-Regulatory Changes.” Current Biology 21 (8): 700–704. doi:10.1016/j.cub.2011.03.028.
- Coyne, J. A., and H. A. Orr. 2004. Speciation. Sinauer Sunderland, MA.
- Darwin, Charles. 1859. On the Origin of Species by Means of Natural Selection or the Preservation of Favoured Races in the Struggle for Life. London: J. Murray.
- Dawkins, Richard. 1976. The Selfish Gene. New York: Oxford University Press.
- Dawkins, Richard. 2016. The Extended Phenotype: The Long Reach of the Gene. Oxford University Press.
- Degnan, James H., and Noah A. Rosenberg. 2009. “Gene Tree Discordance, Phylogenetic Inference and the Multispecies Coalescent.” Trends in Ecology & Evolution 24 (6): 332–40. doi:10.1016/j.tree.2009.01.009.
- de Queiroz, Kevin de. 1999. “The General Lineage Concept of Species and the Defining Properties of the Species Category.” In Species: New Interdisciplinary Essays, edited by Robert A. Wilson, 49–89. Cambridge, MA: MIT Press.
- DiMichele, W. A., A. K. Behrensmeyer, T. D. Olszewski, C. C. Labandeira, J. M. Pandolfi, S. L. Wing, and R. Bobe. 2004. “Long-Term Stasis in Ecological Assemblages: Evidence from the Fossil Record.” Annual Review of Ecology, Evolution, and Systematics 35 (1): 285–322. doi:10.1146/annurev.ecolsys.35.120202.110110.
- Doolittle, W. Ford. 2017. “Making the Most of Clade Selection.” Philosophy of Science 84 (2): 275–95. doi:10.1086/690719.
- Ferris, Kathleen G., Jason P. Sexton, and John H. Willis. 2014. “Speciation on a Local Geographic Scale: The Evolution of a Rare Rock Outcrop Specialist in Mimulus.” Phil. Trans. R. Soc. B 369 (1648): 20140001. doi:10.1098/rstb.2014.0001.
- Fishman, L., J. H. Willis, C. A. Wu, and Y.-W. Lee. 2014. “Comparative Linkage Maps Suggest That Fission, Not Polyploidy, Underlies Near-doubling of Chromosome Number within Monkeyflowers (Mimulus; Phrymaceae).” Heredity 112 (5): 562. doi:10.1038/hdy.2013.143.
- Garner, Austin G., Amanda M. Kenney, Lila Fishman, and Andrea L. Sweigart. 2016. “Genetic Loci with Parent-of-origin Effects Cause Hybrid Seed Lethality in Crosses between Mimulus Species.” New Phytologist 211 (1): 319–31. doi:10.1111/nph.13897.
- Ghalambor, C. K., J. K. McKay, S. P. Carroll, and D. N. Reznick. 2007. “Adaptive versus Non-Adaptive Phenotypic Plasticity and the Potential for Contemporary Adaptation in New Environments.” Functional Ecology 21 (3): 394–407. 10.1111/j.1365-2435.2007.01283.x.
- Ghiselin, Michael T. 1974. “A Radical Solution to the Species Problem.” Systematic Biology 23 (4): 536–44. doi:10.1093/sysbio/23.4.536.
- Gillespie, Rosemary. 2004. “Community Assembly through Adaptive Radiation in Hawaiian Spiders.” Science 303 (5656): 356–59. doi:10.1126/science.1091875.
- Godfrey-Smith, Peter. 2009. Darwinian Populations and Natural Selection. New York: Oxford University Press.
- Gomulkiewicz, R., R. D. Holt, and M. Barfield. 1999. “The Effects of Density Dependence and Immigration on Local Adaptation and Niche Evolution in a Black-Hole Sink Environment.” Theoretical Population Biology 55 (3): 283–96. doi:10.1006/tpbi.1998.1405.
- Gould, S. J., and R. C. Lewontin. 1979. “The Spandrels of San Marco and the Panglossian Paradigm: A Critique of the Adaptationist Programme.” Proceedings of the Royal Society of London. Series B, Biological Sciences 205: 581–98. 10.1098/rspb.1979.0086.
- Gould, S. J., and Elisabeth A. Lloyd. 1999. “Individuality and Adaptation across Levels of Selection: How Shall We Name and Generalize the Unit of Darwinism?” Proceedings of the National Academy of Sciences 96 (21): 11904–9. doi:10.1073/pnas.96.21.11904.
- Gourbière, Sébastien, and James Mallet. 2010. “Are Species Real? The Shape of the Species Boundary with Exponential Failure, Reinforcement, and the ‘Missing Snowball.’ ” Evolution 64 (1): 1–24. doi:10.1111/j.1558-5646.2009.00844.x.
- Grossenbacher, Dena L., Samuel D. Veloz, and Jason P. Sexton. 2014. “Niche and Range Size Patterns Suggest That Speciation Begins in Small, Ecologically Diverged Populations in North American Monkeyflowers (Mimulus Spp.).” Evolution 68 (5): 1270–80. doi:10.1111/evo.12355.
- Haber, Matthew H., and Andrew Hamilton. 2005. “Coherence, Consistency, and Cohesion: Clade Selection in Okasha and Beyond.” Philosophy of Science 72 (5): 1026–40. doi:10.1086/508101.
- Hamilton, Andrew, and Matthew H. Haber. 2006. “Clades Are Reproducers.” Biological Theory 1 (4): 381–91. doi:10.1162/biot.2006.1.4.381.
- Hereford, Joe. 2009. “A Quantitative Survey of Local Adaptation and Fitness Trade-Offs.” The American Naturalist 173 (5): 579–88. doi:10.1086/593131.
- Hull, David L. 1976. “Are Species Really Individuals?” Systematic Biology 25 (2): 174–91. doi:10.2307/2412744.
- Hull, David L. 1980. “Individuality and Selection.” Annual Review of Ecology and Systematics 11: 311–32. 10.1146/annurev.es.11.110180.001523.
- Hutchinson, G. E. 1957. “Concluding Remarks.” In Cold Spring Harbor Symposium on Quantitative Biology, 22:415–427. 10.1101/SQB.1957.022.01.039.
- Janz, N., and S. Nylin. 2008. “The Oscillation Hypothesis of Host-Plant Range and Speciation.” In Specialization, Speciation and Radiation: The Evolutionary Biology of Herbivorous Insects, edited by K. J. Tilmon, 203–15. Berkeley: University of California Press.
- Jorgensen, Tove H., and Jens M. Olesen. 2001. “Adaptive Radiation of Island Plants: Evidence from Aeonium (Crassulaceae) of the Canary Islands.” Perspectives in Plant Ecology, Evolution and Systematics 4 (1): 29–42. doi:10.1078/1433-8319-00013.
- Kinsey, Alfred Charles, Wardell Baxter Pomeroy, Clyde Eugene Martin, and Sam Sloan. 1948. Sexual Behavior in the Human Male. Philadelphia: W. B. Saunders Co.
- Laland, Kevin N., Tobias Uller, Marcus W. Feldman, Kim Sterelny, Gerd B. Müller, Armin Moczek, Eva Jablonka, and John Odling-Smee. 2015. “The Extended Evolutionary Synthesis: Its Structure, Assumptions and Predictions.” Proc. R. Soc. B 282 (1813): 20151019. doi:10.1098/rspb.2015.1019.
- Lavergne, S., and J. Molofsky. 2007. “Increased Genetic Variation and Evolutionary Potential Drive the Success of an Invasive Grass.” Proceedings of the National Academy of Sciences 104 (10): 3883–3888. 10.1073/pnas.0607324104.
- Lesica, Peter, and Fred W. Allendorf. 1995. “When Are Peripheral Populations Valuable for Conservation?” Conservation Biology 9 (4): 753–60. 10.1046/j.1523-1739.1995.09040753.x.
- Lloyd, Elizabeth. 2017. “Units and Levels of Selection.” In The Stanford Encyclopedia of Philosophy, Summer 2017 edition, edited by Edward N. Zalta. Stanford University. https://plato.stanford.edu/archives/sum2017/entries/selection-units/.
- Lomolino, Mark, Brett R. Riddle, and James H. Brown. 2005. Biogeography. 3rd ed.. Sunderland, MA: Sinauer Associates.
- Macnair, M. R. 1989. “A New Species of Mimulus Endemic to Copper Mines in California.” Botanical Journal of the Linnean Society 100 (1): 1–14. 10.1111/j.1095-8339.1989.tb01707.x.
- Martin, Noland H., and John H. Willis. 2007. “Ecological Divergence Associated with Mating System Causes Nearly Complete Reproductive Isolation between Sympatric Mimulus Species.” Evolution 61 (1): 68–82. 10.1111/j.1558-5646.2007.00006.x.
- Mayr, E. 1942. Systematics and the Origin of Species. New York: Columbia University Press.
- Mayr, Ernst. 1982. “Speciation and Macroevolution.” Evolution 36 (6): 1119–32. doi:10.1111/j.1558-5646.1982.tb05483.x.
- McDonald, John H., and Martin Kreitman. 1991. “Adaptive Protein Evolution at the Adh Locus in Drosophila.” Nature 351 (6328): 652. doi:10.1038/351652a0.
- Mishler, Brent D., and John S. Wilkins. 2018. “The Hunting of the SNaRC: A Snarky Solution to the Species Problem.” Philosophy, Theory, and Practice in Biology 10 (1). 10.3998/ptpbio.16039257.0010.001.
- Moczek, Armin P., Karen E. Sears, Angelika Stollewerk, Patricia J. Wittkopp, Pamela Diggle, Ian Dworkin, Cristina Ledon-Rettig, et al. 2015. “The Significance and Scope of Evolutionary Developmental Biology: A Vision for the 21st Century.” Evolution & Development 17 (3): 198–219. doi:10.1111/ede.12125.
- Nixon, Kevin C., and Quentin D. Wheeler. 1992. “Extinction and the Origin of Species.” In Extinction and Phylogeny, edited by Michael J. Novacek and Quentin D. Wheeler, 119–43. New York: Columbia University Press.
- Nosil, Patrik. 2012. Ecological Speciation. Oxford: Oxford University Press.
- Oneal, Elen, John H. Willis, and Robert G. Franks. 2016. “Disruption of Endosperm Development Is a Major Cause of Hybrid Seed Inviability between Mimulus guttatus and Mimulus nudatus.” The New Phytologist 210 (3): 1107–20. doi:10.1111/nph.13842.
- Orzack, Steven Hecht, and Patrick Forber. 2017. “Adaptationism.” In The Stanford Encyclopedia of Philosophy, edited by Edward N. Zalta, Spring 2017 edition. https://plato.stanford.edu/archives/spr2017/entries/adaptationism/.
- Oskolski, Alexei. 2011. “The Taxon as an Ontological Problem.” Biosemiotics 4 (2): 201–22. doi:10.1007/s12304-010-9099-4.
- Palumbi, Stephen R. 2001. “Humans as the World’s Greatest Evolutionary Force.” Science 293 (5536): 1786–90. 10.1126/science.293.5536.1786 .
- Phillips, B. L., G. P. Brown, J. K. Webb, and R. Shine. 2006. “Invasion and the Evolution of Speed in Toads.” Nature 439 (7078): 803–803. doi:10.1038/439803a.
- Polechová, Jitka, and Nicholas H. Barton. 2015. “Limits to Adaptation along Environmental Gradients.” Proceedings of the National Academy of Sciences 112 (20): 6401–6. doi:10.1073/pnas.1421515112.
- Potochnik, Angela, and Brian McGill. 2012. “The Limitations of Hierarchical Organization.” Philosophy of Science 79: 120–40. doi:10.1086/663237.
- Pyron, R. Alexander, Gabriel C. Costa, Michael A. Patten, and Frank T. Burbrink. 2015. “Phylogenetic Niche Conservatism and the Evolutionary Basis of Ecological Speciation.” Biological Reviews 90 (4): 1248–62. doi:10.1111/brv.12154.
- Rieppel, Olivier. 2011. “Species Are Individuals—the German Tradition.” Cladistics 27 (6): 629–45. doi:10.1111/j.1096-0031.2011.00356.x.
- Rieppel, Olivier. 2013. “Biological Individuals and Natural Kinds.” Biological Theory 7 (2): 162–69. doi:10.1007/s13752-012-0051-5.
- Rieseberg, Loren H., Margaret A. Archer, and Robert K. Wayne. 1999. “Transgressive Segregation, Adaptation and Speciation.” Heredity 83 (4): 363–72. doi:10.1038/sj.hdy.6886170.
- Rosenberg, Alexander. 2006. Darwinian Reductionism: Or, How to Stop Worrying and Love Molecular Biology. Chigago: University of Chicago Press.
- Sanford, E., and M. W. Kelly. 2011. “Local Adaptation in Marine Invertebrates.” Annual Review of Marine Science 3: 509–35. doi.org/10.1146/annurev-marine-120709-142756.
- Schluter, Dolph. 2000. The Ecology of Adaptive Radiation. Oxford, UK: Oxford University Press.
- Seehausen, Ole. 2006. “African Cichlid Fish: A Model System in Adaptive Radiation Research.” Proceedings of the Royal Society of London B: Biological Sciences 273 (1597): 1987–98. doi:10.1098/rspb.2006.3539.
- Sexton, Jason P., Jorge Montiel, Jackie E. Shay, Molly R. Stephens, and Rachel A. Slatyer. 2017. “Evolution of Ecological Niche Breadth.” Annual Review of Ecology, Evolution, and Systematics 48 (1): 183–206. doi:10.1146/annurev-ecolsys-110316-023003.
- Shaw, Kerry L., and Sean P. Mullen. 2014. “Speciation Continuum.” Journal of Heredity 105 (S1): 741–42. doi:10.1093/jhered/esu060.
- Simón-Porcaré, Violeta I., Jose L. Silva, Sofie Meeus, James D. Higgins, and Mario Vallejo-Marín. 2017. “Recent Autopolyploidization in a Naturalized Population of Mimulus guttatus (Phrymaceae).” Botanical Journal of the Linnean Society 185 (2): 189–207. doi:10.1093/botlinnean/box052.
- Smith, Thomas B., Michael T. Kinnison, Sharon Y. Strauss, Trevon L. Fuller, and Scott P. Carroll. 2014. “Prescriptive Evolution to Conserve and Manage Biodiversity.” Annual Review of Ecology, Evolution, and Systematics 45 (1): 1–22. doi:10.1146/annurev-ecolsys-120213-091747.
- Sober, Elliott, and David Sloan Wilson. 1994. “A Critical Review of Philosophical Work on the Units of Selection Problem.” Philosophy of Science 61 (4): 534–55. doi:10.1086/289821.
- Stegenga, Jacob. 2016. “Population Pluralism and Natural Selection.” The British Journal for the Philosophy of Science 67 (1): 1–29. doi:10.1093/bjps/axu003.
- Sweigart, A. L., N. H. Martin, and J. H. Willis. 2008. “Patterns of Nucleotide Variation and Reproductive Isolation between a Mimulus Allotetraploid and Its Progenitor Species.” Molecular Ecology 17 (8): 2089–2100. doi:10.1111/j.1365-294X.2008.03707.x.
- Takahashi, Yuma, Yoshihisa Suyama, Yu Matsuki, Ryo Funayama, Keiko Nakayama, and Masakado Kawata. 2016. “Lack of Genetic Variation Prevents Adaptation at the Geographic Range Margin in a Damselfly.” Molecular Ecology 25 (18): 4450–60. doi:10.1111/mec.13782.
- Theobald, Douglas L. 2010. “A Formal Test of the Theory of Universal Common Ancestry.” Nature 465 (7295): 219. doi:10.1038/nature09014.
- Thomas, Christopher M., and Kaare M. Nielsen. 2005. “Mechanisms of, and Barriers to, Horizontal Gene Transfer between Bacteria.” Nature Reviews Microbiology 3 (9): 711–21. doi:10.1038/nrmicro1234.
- Thornhill, Andrew H., Bruce G. Baldwin, William A. Freyman, Sonia Nosratinia, Matthew M. Kling, Naia Morueta-Holme, Thomas P. Madsen, David D. Ackerly, and Brent D. Mishler. 2017. “Spatial Phylogenetics of the Native California Flora.” BMC Biology 15: 96. doi:10.1186/s12915-017-0435-x.
- Vallejo-Marín, Mario, Richard J. A. Buggs, Arielle M. Cooley, and Joshua R. Puzey. 2015. “Speciation by Genome Duplication: Repeated Origins and Genomic Composition of the Recently Formed Allopolyploid Species Mimulus Peregrinus.” Evolution 69 (6): 1487–1500. doi:10.1111/evo.12678.
- Vellend, Mark, Lander Baeten, Antoine Becker-Scarpitta, Véronique Boucher-Lalonde, Jenny L. McCune, Julie Messier, Isla H. Myers-Smith, and Dov F. Sax. 2017. “Plant Biodiversity Change across Scales during the Anthropocene.” Annual Review of Plant Biology 68 (1): 563–86. doi:10.1146/annurev-arplant-042916-040949.
- West-Eberhard, Mary Jane. 2005. “Developmental Plasticity and the Origin of Species Differences.” Proceedings of the National Academy of Sciences 102 (suppl 1): 6543–49. doi:10.1073/pnas.0501844102.
- Wiens, John J. 2004. “Speciation and Ecology Revisited: Phylogenetic Niche Conservatism and the Origin of Species.” Evolution 58 (1): 193–97. 10.1111/j.0014-3820.2004.tb01586.x.
- Wiens, John J., and Catherine H. Graham. 2005. “Niche Conservatism: Integrating Evolution, Ecology, and Conservation Biology.” Annual Review of Ecology, Evolution, and Systematics 36 (1): 519–39. doi:10.1146/annurev.ecolsys.36.102803.095431.
- Wu, C. A., D. B. Lowry, A. M. Cooley, K. M. Wright, Y. W. Lee, and J. H. Willis. 2007. “Mimulus Is an Emerging Model System for the Integration of Ecological and Genomic Studies.” Heredity 100: 220–30. 10.1038/sj.hdy.6801018.
- Zhang, Jianzhi. 2018. “Neutral Theory and Phenotypic Evolution.” Edited by Sudhir Kumar. Molecular Biology and Evolution 35 (6): 1327–31. doi:10.1093/molbev/msy065.
Acknowledgments
I thank Ford Doolittle, Quentin Wheeler, and many other attendees of the “Species in the Age of Discordance” Meeting at the University of Utah (23–25 March 2017) for valuable discussions. I thank Matt Haber and two anonymous reviewers for comments that greatly improved the manuscript. This work was supported by a grant from the Hellman Fellows Fund, and by grant number 1557117 from the National Science Foundation.
Copyright © 2019 Author(s)
This is an open-access article distributed under the terms of the Creative Commons Attribution 4.0 International license, which permits anyone to download, copy, distribute, display, or adapt the text without asking for permission, provided that the creator(s) are given full credit.
ISSN 2475-3025